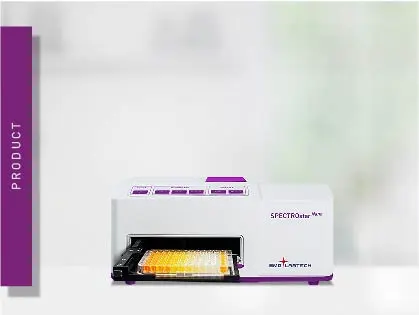
SPECTROstar Nano
Absorbance plate reader with cuvette port
Cell metabolism is the sum of all chemical and biochemical reactions that take place in biology to sustain life, allowing organisms to maintain their structures, grow, proliferate, and react to environmental changes. The main function of metabolism is the conversion of nutrients into energy to run cellular processes, into basic molecules to be used as building blocks for cell growth, and the elimination of useless by-products.
In biology, the word “metabolism” also refers to the process of digestion and the absorption and transport of nutrients to and among tissues and cells. This blog post focuses mainly on metabolic processes happening in eukaryotic cells but you can read more about bacterial metabolism here.
Cell metabolism encompasses different steps and sequences of molecular conversions. This coordinated series of biochemical reactions where the product of one conversion becomes the substrate for another reaction is known as metabolic pathways. In these pathways, molecules are transformed by a series of enzymes.
Basic metabolism can be simplified to pathways focusing on the use of major nutrients such as glucose, amino acids and fatty acids which are fundamental for energy production, homeostasis and growth (Fig. 1).
In biology, scientific research on cell metabolism focuses on different topics such as understanding the fundamental metabolic processes, metabolic changes and their role in diseases (including cancer cell and tumor metabolism), the development of therapies, the identification of unknown metabolic processes and metabolites, etc. However, this is complicated by the large size of metabolic networks and reactions. As today, more than 8,700 metabolic reactions and 16,000 metabolites are annotated.1
Metabolic reactions are interconnected and produce and recycle the components required for fundamental activities like cell growth (e.g., nucleotides, amino acids, Adenosine triphosphate - ATP) and signaling (e.g., cAMP). In general, metabolic reactions can be divided into catabolic and anabolic processes with the former releasing energy and the latter consuming it (fig.2). Metabolism also includes the disposal of waste and by-products.
Anabolism is a set of constructive processes and pathways that synthesise simple molecules and polymerize them into macromolecules (e.g., nucleic acids, enzymes, etc.). In the cell, these macromolecules are synthesized in a step-by-step process from simple precursors. Anabolism consists of three basic steps: the production of precursor molecules (e.g., nucleotides, monosaccharides, etc.), their activation, and their assembly into macromolecules and polymers (e.g., DNA, proteins, polysaccharides, etc.). Cells require energy for this process.2
Organisms are categorised based on their anabolism and according to the source used by cells to build-up molecules. Autotroph organisms (e.g., plants) produce the required macromolecules from simple precursors like CO2 and H2O, whereas heterotrophs require a source of more complex substances (e.g., monosaccharides like glucose).
In carbohydrate anabolism, organic acids are converted into monosaccharides (e.g., glucose) and finally assembled to complex sugars (polysaccharides). Gluconeogenesis, the generation of glucose, enzymatically converts pyruvate to glucose-6-phosphate (fig. 3).3 Glucose is then stored as an energy resource in most tissues or as fat. In vertebrates, the fatty acids though cannot be converted to glucose by gluconeogenesis as these organisms do not have the necessary enzymatic machinery, as opposed to plants and bacteria.4 Alternatively, the metabolite acetyl CoA is again fed into the Tricarboxylic acid (TCA) cycle for energy production.
Polysaccharides are made by the sequential addition of monosaccharides by glycosyltransferase and result in straight or branched structures. These complex sugars can then be transferred to lipids and proteins or used for metabolic or structural purposes.5
Glucose is used as a substrate for glycogenesis, the synthesis of glycogen, a multibranched glucose polysaccharide. Glycogen serves as short-term energy reserve compared to fat deposits and is mainly stored in liver and muscle cells.
Proteins are made of amino acids linked by a chain of peptidic bonds. Amino acids are synthesized from intermediate products of glycolysis, the TCA cycle (tricarboxylic acid cycle), or the pentose phosphate pathway. Organisms vary in their metabolic capability to synthesize amino acids. While bacteria and plants can synthesize all twenty, mammals are limited to the eleven non-essential ones, the remaining nine essential ones must be gained from food.6
Amino acids are also used as a source of nucleotide precursors. Adenine and guanine are synthesized as nucleosides from the precursor nucleoside inosine monophosphate. Cytosine and thymine origin from glutamine and aspartate. Nucleotides are synthesized in pathways that require large amounts of energy. Fatty acids instead are polymerized by enzymes called fatty acid synthases. These extend the acyl chains of the fatty acids in a reaction cycle.
Photosynthesis encompasses all metabolic pathways that enable carbon fixation and the synthesis of carbohydrates from CO2 and sunlight. This pathway is used by plants, cyanobacteria and algae. In the Calvin cycle, this process uses ATP and NADPH to convert CO2 to glycerate 3-phosphate and ultimately to glucose (fig.4). There are three different types of photosynthesis in plants: C3 carbon fixation, C4 carbon fixation and Crassulacean acid metabolism (CAM) photosynthesis. They differ by the path that CO2 takes to the Calvin cycle.
Catabolic pathways are used by cells to break down and degrade molecules and macromolecules. The final purpose of catabolism is to produce energy and the basic components required by anabolism. Depending on the used substrates, reactions can be divided in carbohydrate, protein, and fat catabolism.
Organisms can be divided in different groups based on their catabolism which determines whether specific substances are used as nutrients or are rather toxic. Organotrophs degrade organic molecules, lithotrophs use inorganic components, phototrophs convert sunlight to chemical energy, whereas chemotrophs depend on redox reactions. Catabolic reactions are typically exothermic.
In eukaryotes, proteins, carbohydrates and lipids are the main fuel molecules. Besides the digestion taking place in the alimentary tract of multicellular organisms, catabolic reactions include three main steps. Outside of the cell, large organic molecules (e.g., polysaccharides, lipids and proteins) are broken down into smaller components without any energy output. These are taken up and converted by cells to smaller molecules (usually acetyl coenzyme A; acetyl-CoA). This process produces energy. In the third step, acetyl-CoA is oxidized to water and CO2. This happens in the citric acid cycle and in the electron transport chain of mitochondria. This step produces a larger amount of energy while reducing NAD+ to NADH.7
In carbohydrate catabolism, polymeric carbohydrates like glycogen are first broken down to smaller components such as monosaccharides. These are typically degraded by glycolysis with conversion of glucose and fructose to pyruvate and energy (ATP; fig. 5).
Pyruvate then is converted to acetyl-CoA through aerobic glycolysis and can then enter the TCA-cycle which produces some more ATP molecules (fig. 6).
The most important product of these steps though is NADH as it is used by the electron transport chain in the inner mitochondrial membrane of eukaryotes (fig.7). Oxidative phosphorylation is used here to oxidize substrates: the electrons removed from organic molecules in previously mentioned pathways are transferred to oxygen and the resulting released energy is used to produce a multiple of the amount of ATP previously made available during glycolysis.
In the mitochondria, a proton concentration difference across the membrane is created by pumping protons out of the organelles. This creates an electrochemical gradient that drives protons back into the mitochondrion through the enzyme ATP synthase. Through this enzyme, the flow of protons can be used to phosphorylate adenosine diphosphate to the highly energetic ATP.8
Anaerobic respiration is used when only limited amounts of oxygen are available. Under these conditions, depending on the organism lactate and/or ethanol are produced from the pyruvate generated during glycolysis (fig. 8). Though much faster than aerobic respiration, it yields a much lower amount of ATP molecules.
Fats are degraded to free fatty acids and glycerol by hydrolysis. Fatty acids are further catabolised by beta oxidation to acetyl-CoA. Glycerol is degraded by glycolysis. Of notice, fatty acids generate more energy than carbohydrates. Amino acids can be oxidised to urea and CO2 to produce energy.
Adenosine triphosphate (ATP) is a central co-enzyme and the main cellular energy carrier. In fact, the energy released when the chemical bonds of nutrients are broken is mainly captured in ATP molecules (fig. 9). Although in cells there are only small amounts of ATP, it is continuously degraded and regenerated. Actually, an adult human body consumes its own weight in ATP in a single day.8 Catabolism generates ATP while anabolism consumes it.
ATP can be synthesised in two ways. The first is oxidative phosphorylation in mitochondria. Oxidative phosphorylation is the predominant pathway of ATP synthesis in most eukaryotic cells and involves ATP synthesis from ADP and phosphate. In the second process, ATP is produced via the transfer of high-energy phosphoryl groups from energy molecules to ADP. This process takes place during glycolysis and during the TCA cycle. Per unit of glucose, glycolysis is an inefficient means of generating ATP compared to the amount obtained by mitochondrial respiration.
Plants and different bacteria take their energy from sunlight. Typically, the conversion of solar light into energy is similar to oxidative phosphorylation as energy is stored as a proton concentration gradient that drives ATP production.9 The main difference is that the electrons needed come from light-gathering proteins (fig. 10).
Metabolic by-products are waste substances that cannot be further processed by the organism and are partly toxic in high quantities. Therefore, they have to be excreted from the cells, tissues and finally organism to maintain cellular health and homeostasis. The most common waste products include water, CO2 and nitrogen compounds. Besides CO2, which is excreted by the lungs, in complex organisms metabolic waste is typically excreted through the kidneys as water solute.
Reactive oxygen species (ROS) are partially reduced metabolites of oxygen and by-products of aerobic metabolism, cellular respiration and photosynthesis in mitochondria, peroxisomes and chloroplasts. They are strongly oxidizing and, at high concentrations, are deleterious to cells damaging DNA, proteins and lipids, and eventually leading to cell death. However, at low concentrations, they exert signaling and gene expression functions. For instance, hydrogen peroxide also acts as a second messenger in different signaling pathways.
To maintain ROS at low levels, cells exploit different antioxidant systems. For instance, NADPH generated during glucose metabolism is an important redox cofactor for the antioxidant enzyme ‘glutathione reductase’. This shows how cell metabolism is strongly interlinked to the redox state.
Metabolic perturbations and metabolic reprogramming are associated with common human diseases. In fact, metabolism should not be considered as a self-regulating entity that is independent of other biological pathways. On the contrary, cell metabolism affects or is affected by a multitude of processes. This is even more evident considering the fact that, during the course of evolution, fundamental energy metabolism pathways have not been modified and still are conserved throughout vastly different species. For instance, intermediates of the TCA cycle are present in all known organisms from unicellular bacteria to complex and large multicellular organisms like elephants.10 Metabolism is evidently perturbed in different diseases like cancer, diabetes, and metabolic syndrome.
A modified cell metabolism supports the malignant transformation in cancer, as well as the growth and preservation of tumors. It is therefore considered a hallmark of cancer.11 Cancer cells typically outrun surrounding cells for nutrients (e.g., glucose). A widespread hypothesis is that aggressive rapid cancer cell growth and its associated increased bioenergetic demands force cancer cells to a metabolic reprogramming of their pathways.12
This is mainly described as the Warburg effect from German scientist Otto Warburg, who was awarded the Nobel Prize in Physiology in 1931. Warburg´s fundamental observation was that cancer cell metabolism typically produces energy through the less efficient glycolysis pathway instead of the most efficient TCA cycle and oxidative phosphorylation pathways, as normal cells do (fig. 11).
In fact, even in the presence of oxygen, cancer cells rely mainly on anaerobic lactic acid fermentation taking place in the cytosol. The detailed mechanism of cancer cell metabolism and of the Warburg effect and its therapeutic implications are still unclear. In addition, cancer cells also typically display enhanced ROS levels.
The aberrant cancer metabolism can be exploited in diagnosis and therapy. In diagnostics, the increased glucose metabolism of cancer cells is used in positron emission tomography (PET) scans. In anti-tumor therapy development, this effect is a main focus of drug discovery.
In biology, metabolic readouts can be used as key molecular indicators of healthy cellular functions or as predictors of disease (e.g., cancer, diabetes, etc.). These readouts also play a role in drug discovery. Here, the drug-mediated “adjustment” of metabolic pathways (metabolic reprogramming) can be used to restore a healthy phenotype to enhance cell performance, or to specifically target and kill cancer.
In biology and life science applications, basic molecular analysis of cell metabolism typically focusses on the two main pathways: glycolysis and mitochondrial respiration. These play a critical role in different molecular processes and can be used as indicators of cell health and disease, as well as of cell proliferation, death and homeostasis.
Modern microplate readers play an important role as they enable the analysis of different metabolic parameters in higher throughput and in an automated manner.
In addition, the dedicated cell-culture features of BMG LABTECH plate readers enable the monitoring of metabolic activity in real-time in live cells and with more physiological conditions, providing important details about cell homeostasis, mitochondrial function, and substrate and energy consumption.
Metabolic microplate-based assays can be divided in two categories: the ones that exploit metabolic functions as an indirect readout and the ones that directly target specific pathways. For the former, enzymatic pathways as well as ATP presence are exploited as readout to analyse different functions in cells. An active metabolism is a sign of viable cells and can be hence exploited by viability assays (fig.12) as described in the app notes Viability assays: a comparison of luminescence-, fluorescence-, and absorbance-based assays to determine viable cell counts and The new Atmospheric Control Unit (ACU) for the CLARIOstar provides versatility in long-term cell-based assays. In addition, ATP is used as fuel by many luciferase-based assays.
Direct metabolic readouts focus mainly on glucose consumption, mitochondrial function, glycolytic activity and generation of by-products (radical oxygen species). Glucose presence in the cell culture medium and its consumption by cells over time can also be assessed by luminescence, as shown in the app note Glucose assay and lactate assay allow to monitor cellular glucose metabolism precisely.
Mitochondrial function is commonly evaluated through the analysis of oxygen consumption. In the app note Real-time monitoring of intracellular oxygen using MitoXpress-Intra, the MitoXpress Intra assay is used to measure oxygen consumption with an oxygen-sensitive fluorescent probe. As cells respire, the depletion of oxygen within a cell monolayer is analysed kinetically in real-time on a CLARIOstar® Plus microplate reader with Atmospheric Control Unit (ACU). The ability of the CLARIOstar Plus with ACU to run gas ramps also enables studies in hypoxic environments as shown in the app note The CLARIOstar with ACU exposes cells to ischemia-reperfusion conditions and monitors their oxygenation (fig. 13). These assays can also be used for safety assessments as well screenings for drug-induced metabolic side effects and mitochondrial dysfunction. This can considerably influence organ toxicity for instance in the heart, liver and kidney. NADH/NAD+ and NADPH/NADP+ are cofactors used by many enzymes in metabolism and for different mitochondrial functions. The reduction of NAD+ to NADH and NADP+ to NADPH can be easily monitored in absorbance as their oxidized forms do not absorb light at 340 nm (fig. 14).
Glycolytic activity under anaerobic conditions is typically evaluated by means of the analysis of extracellular acidification. The conversion of pyruvate to lactate and its secretion in the extracellular environment thereby leads to the extracellular acidification of the cell culture medium. In cancer cells, lactate presence can be used as a marker of disease.
Lactate is quantified by time-resolved fluorescence in the app note Assessment of extracellular acidification using a fluorescence-based assay. Another luminescence-based method is shown in the app note Glucose assay and lactate assay allow to monitor cellular glucose metabolism precisely.
Cellular redox changes can be monitored in different ways. One approach is to use genetically encoded biosensors based on redox-sensitive GFP (roGFP). Further, the following blog post discusses ROS detection in general.
Absorbance plate reader with cuvette port
Powerful and most sensitive HTS plate reader
Most flexible Plate Reader for Assay Development
Upgradeable single and multi-mode microplate reader series
Flexible microplate reader with simplified workflows
Learn about applications for bacterial metabolism on a microplate reader.
Mitochondrial toxicity can have devastating effects on the cell and life. Find out how microplate readers can be used to assess mitochondrial health and how this impacts disease and drug discovery.
Reactive oxygen species (ROS) may have physiological as well as pathological effects. Here we explain what ROS are and how they can be measured on microplate readers.
Redox processes play an important role in cell homeostasis. Read here, how to monitor cellular redox changes with roGFP using the most sensitive, high-performance plate readers from BMG LABTECH.
The pH-Xtra assay is a TRF-based assay to assess extracellular acidification as a measure of glycolytic activity. Read more about the assay and its performance here.