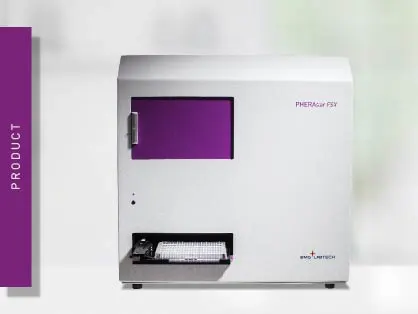
PHERAstar FSX
Powerful and most sensitive HTS plate reader
Enhance assay sensitivity with time-resolved fluorescence, reducing background noise for accurate immunoassays and interaction studies.
Fluorescence is the emission of light by a molecule upon excitation by light at a shorter wavelength than the emitted one.
Fluorescence detection can be mostly divided into two types of measurements: steady-state and time-resolved fluorescence. The main difference between these two methods is based on the nature and properties of the fluorescent molecules (fluorophores) used and the consequent time of detection.
Steady-state is the most widespread fluorescent detection mode and is commonly referred to as “fluorescence intensity”. Standard fluorophores (e.g. fluorescein, rhodamine, etc.) emit light at a specific wavelength within nanoseconds upon excitation. This very short time gap between excitation and emission allows the almost concomitant detection of the fluorescent emission signal with the excitation of the sample.
The second type, time-resolved fluorescence (TRF), is monitored as a function of time upon excitation. In contrast to steady-state fluorescence intensity, time-resolved fluorescence is based on the detection of intensity decays and/or on the delayed detection of the emission signal upon excitation. In time-resolved fluorescence measurements, the excitation light pulse is shorter than the decay time of the fluorescent signal. Time-resolved fluorescence detection can only be achieved when the emission signal of the fluorophore is prolonged to the micro- or even milli-second range and not short-lived within nanoseconds as for common labels (fig.1).
Lanthanides (Ln) are a group of uniquely fluorescent metallic chemical elements often collectively known as “rare earth elements”. Lanthanides have very low absorption (excitation) coefficients and slow emission rates. This results in prolonged fluorescence decay times between 0.5 to 3 milliseconds (long lifetimes). All lanthanide elements form trivalent cations (Ln3+) and display emission in an aqueous solution. Additionally, their emission peaks are very sharp and narrow with a large Stokes shift.[1]
Lanthanides possess favorable properties as biochemical probes. Originally, they have been employed in biological systems as luminescent probes for calcium. In fact, lanthanide luminescence has been demonstrated to be a sensitive sensor for Ca2+ binding sites in proteins.[2]
Their prolonged fluorescence decay makes them ideal fluorophores for time-resolved fluorescence applications. Four of them, europium, terbium, samarium, and dysprosium have found widespread use in the life sciences, in particular in time-resolved fluorescence immunoassays, with europium and terbium being the most commonly used (fig.2).
Europium ions (Eu3+) in particular, are frequently used as labels for time-resolved fluorescence detection in immunological assays. Besides the long emitting fluorescence, europium displays a large Stokes shift (290 nm) with no overlap between the excitation and emission spectrum, and with only 10 nm bandwidth, a very sharp emission spectrum at 615 nm (fig.3).[3]
As the emission of lanthanides is usually too weak for time-resolved fluorescence applications, they are generally not directly excited, but are usually embedded in a sort of light-collecting “cage”. This cage, most commonly a chelate or a cryptate, allows both energy collection and energy transfer to the lanthanide ions, resulting in higher emission intensities (fig.4). It is worth to be mentioned that the excitation spectrum of the cage-lanthanide complex reflects the absorption spectrum of the “cage” and not of the lanthanide itself.[4]
In addition to a higher emission signal, chelation makes lanthanide ion conjugation to biological components (e.g. antibodies, receptors, ligands, etc.) possible, a mandatory requirement for several time-resolved fluorescence applications.
Similar to fluorescence intensity detection, the setup for time-resolved fluorescence measurements consists of a light source, an optical device for wavelength selection, and a photomultiplier tube (PMT) detector.
As the lanthanide-chelate/cryptate complex is typically excited at 337 nm, a xenon flash lamp or a specific laser is used as light source for time-resolved fluorescence detection. Multi-mode plate readers are equipped with a broadband xenon flash lamp as it offers more flexibility for multiple detection methods. High-end readers may offer in addition an optional dedicated time-resolved fluorescence excitation laser. The laser focuses more energy on the specific excitation wavelengths of lanthanides and possibly leads to better results with better discrimination between low and high signals. However, given the sharp excitation at around 337 nm, this is just a single-purpose light source that cannot be used as excitation source for other detection methods but time-resolved fluorescence.
Regarding wavelength selection, both filter- and monochromator-based plate readers can be employed for the detection of time-resolved fluorescence assays. As filter-based readers are usually more sensitive than monochromators because of higher light transmission, they are recommended for TRF assays with a limited photon yield. Typically, time-resolved fluorescence energy transfer assays are particularly challenging for monochromator-based readers.
Photomultiplier tubes (PMTs) are used as detectors. Because of the long emitting properties of lanthanides, in time-resolved fluorescence, the PMT detector is turned on after the excitation has occurred. This allows the short-lived autofluorescent signal to fade out. The intensity of the emission signal is then integrated as a function of time for a specific time window. These two parameters are referred to as “integration start” and “integration time” and are usually in the microsecond range (fig. 5).
High-end microplate readers can also be equipped with so-called photon-counting PMTs for time-resolved fluorescence. While normally microplate readers simply give an integration value for the area under the curve during the integration time, time-resolved fluorescence -dedicated photon-counting detection monitors the whole decay curve of the lanthanide.
On the PHERAstar FSX reader, photon-counting detection allows the measurement and display of the emission decay curve with a time resolution of 2 microseconds. This unique feature called Decay Curve Monitoring simplifies time-resolved fluorescence assay development, helping to optimize timing parameters and thus improving signal detection and reducing background noise.
Immunoassays based on time-resolved fluorescence are used to quantify specific molecules such as proteins or cytokines. They rely on target recognition and binding by specific antibodies that have been labelled with a fluorophore. Analysis and quantification of the fluorescent signal indirectly provide information on the target molecules. Immunoassays are quantitative, highly sensitive, and provide the possibility for multi-parameter detection (multiplexing).
Similar to the classical ELISA assays, time-resolved fluorescence immunoassays commonly use a capture antibody bound to the bottom of the microplate well. As the samples are incubated in these wells, the antibody captures the target molecule on the plate. After removal of unbound sample by washing the plate, a second antibody covalently bound to a lanthanide chelate, most commonly europium, is added. This binds the target molecule while non-bound second antibody is washed away. The amount of lanthanide labelled antibody is proportional to the concentration of the target molecule in the sample. Time-resolved fluorescence immunoassays can be performed as direct or competitive assays.
A dissociative enhancement step is required to free the europium molecules from their “cage” on the antibody because of three reasons: first, lanthanides have poor light absorption; second, they are rarely directly excited; and third, lanthanide chelates are only poorly fluorescent when conjugated to biological components (antibodies in this case). The dissociation is promoted by the addition of a specific solution commonly referred to as an “enhancement solution”. Besides dissociating the lanthanide, the solution promotes the formation of a new chelate “cage” that incorporates the chromophore necessary for the excitation of the lanthanide. The chelator-chromophore in the enhancer solution is used to absorb and transfer the excitation light to the lanthanide, significantly increasing the intensity of the emission signal (fig.6).
Samples are excited by a pulse of light at a specific wavelength, usually 337 nm. The detection is time-gated on the decay of the autofluorescence signal. This means that time-resolved fluorescence detection starts only after exhaustion of the short-lived autofluorescence signal (microseconds). The detected emission signal is integrated for a specific time window and data are measured as integrated intensity, not time decay. As the amount of analyte is proportional to the time-resolved emission signal, it can be easily quantitated with the use of a standard curve.
One of the most commonly used time-resolved fluorescence immunoassays is DELFIA®.
DELFIA
DELFIA (Dissociation-Enhanced Lanthanide Fluorescent Immunoassay) is a heterogeneous time-resolved fluorescence wash-based assay developed on a similar principle and workflow as ELISAs. It is said to overcome the typical limitations of ELISA assays, providing a wider dynamic range and stable signals that can be measured up to months after the execution of the assay. For DELFIA detection, plate readers have to be equipped with time-resolved fluorescence detection with excitation at 337 nm and emission at 615 nm.
In DELFIA assays, capture antibodies are bound to the microplate. Upon sample addition and a series of wash steps to eliminate the unbound sample, a europium-labelled detection antibody is added. Finally, an enhancement solution is added after a final series of washes. As mentioned above, DELFIA assays require a dissociative enhancement step to produce a fluorescent signal. This dissociation promotes the formation of a new highly fluorescent chelate in a stable micellar solution. An example of a DELFIA immunoassay is shown in the application note “Time-Resolved Fluorescence (TRF) immunoassay in 384-well format using a matched antibody pair kit and the PHERAstar FSX”.
Although robust and very sensitive, DELFIA assays are not suited for high-throughput screening, as the procedure involves binding, incubation, and washing steps.
Exosome detection
Exosomes are extracellular vesicles of endosomal origin, containing nucleic acids, proteins, and lipids. They facilitate intercellular communication between different cell types within an organism. The TRIFic™ time-resolved fluorescence exosome detection kits detect the presence of exosomes based on the expression of specific membrane proteins.
Cell metabolism
Specific fluorescent oxygen- and pH-sensitive probes are available for the analysis of cellular metabolic parameters in time-resolved fluorescence mode. In specific application notes, we show how intra-cellular and extra-cellular oxygen consumption as well glycolytic activity can be determined in real-time in living cells.
Screening for protein interaction
Probably, the other most popular application of time-resolved fluorescence can be found in TR-FRET (time-resolved fluorescence energy transfer). Its main use is found in drug and high-throughput screening applications as time-resolved fluorescence energy transfer assays are robust and easy to automate and miniaturize. Their main application is protein-protein or ligand-receptor interaction studies.
Reduction of background noise
Autofluorescence is emitted by all biological samples and is usually a limiting factor for assay sensitivity. Since it decays in nanoseconds, time-resolved fluorescence measurements (time-gated) in the micro- or milli-second range allow detecting the emission signal of the lanthanides upon exhaustion of the autofluorescence signal. The “short-lived” fluorescent noise (background signals and scattered excitation light) is eliminated and the time-resolved fluorescence (long-lived) signals can be measured with very high sensitivity, the only background signal being the non-specifically bound label. This reduces background noise and increases sensitivity when compared to steady-state fluorescence intensity detection.
A large Stokes shift
The difference between the excitation and emission wavelength maxima (peaks) is defined as the Stokes shift. For many commercially available steady-state fluorophores, this is relatively narrow and results in self-quenching of the signal due to overlap between absorption and emission spectra. On the contrary, lanthanides have large Stokes shifts, greatly increasing the signal-to-background (S/B) ratio in time-resolved fluorescence detection.
High-intensity signals
Lanthanides have a high quantum yield with a higher fluorescence intensity than “regular” fluorophores. This significantly improves assay sensitivity in time-resolved fluorescence. Of notice, the high quantum yield makes lanthanides perfectly suited as donors but unsuited as acceptors.
Long assay stability
If time-resolved fluorescence immunoassays are stopped before the dissociation/enhancement step and properly stored, the assays are stable for up to 10 years, according to the manufacturers. The only step needed to revive them is the addition of the enhancement solution.
Multiple steps
As discussed above, chelates usually embed lanthanides in “cages” that have the function of stabilizing, binding the fluorophore to a molecule, or enhancing its fluorescent properties. In time-resolved fluorescence detection, this last step is needed to increase the emission intensities that would be quite poor if the lanthanides were in their “natural” state. The switch between these different types of cages provides however a significant limitation, as multiple intermediate steps like washing or addition of solutions are required. This is usually not the case for fluorescence intensity-based assays.
Higher costs
Although more sensitive and efficient, time-resolved fluorescence detection requires a higher expenditure for reagents and instrumentation, when compared to fluorescence intensity methods.
Use the appropriate microplate for time-resolved fluorescence
Time-resolved fluorescence assays usually require detection from the top of the well and are typically run in white plates. As the time-delayed detection eliminates autofluorescence and reduces background, white plates are beneficial. They reflect and enhance the emission of light, providing stronger signals. Black plates are suggested in case a strong signal may saturate the detector.
For time-resolved fluorescence cell-based assays, detection from the bottom of a plate with white/black walls and a clear bottom is recommended. However, as not all clear bottoms are UV transparent, the plastic may absorb light at 340 nm and hence significantly reduce the excitation light (typically at 337 nm). Before running your time-resolved fluorescence assay, it is advisable to check the specifications of your microplate and use plates with a UV-transparent bottom. For more details, read our blog post "The microplate: utility in practice."
Wash thoroughly
As discussed before, time-resolved detection eliminates autofluorescence. However, the washing steps after incubation with lanthanide-labelled reagents and before the addition of the enhancement solution are quite critical and may affect assay quality. These steps have to be very efficient in order to remove all the unbound labelled reagents. If this is not the case, the assay will produce a high background signal caused by the presence of unspecific unbound lanthanide ions.
Use TRIS-HCl instead of phosphate buffers
For DELFIA assays, tris-HCl-based buffers should be preferred over phosphate-based ones. On one hand, high phosphate concentrations can potentially dissociate europium from its chelate during long incubation times. On the other, the maximum signal is generally lower with phosphate-based buffer when compared to tris-HCl-based.
Avoid EDTA and low pH values
Low pH can potentially release the lanthanide ion from its chelate. This can negatively affect the integrity of the dye, and increase non-specific binding and background in time-resolved fluorescence detection. Additionally, beware of the presence of EDTA in your reagents as it can inactivate lanthanide chelates.
No light polarisation
As lanthanides lack polarised light emission, they cannot be used for anisotropy/ fluorescence polarisation measurements.[5]
DELFIA is a registered trademark of Perkin Elmer, Inc.
Powerful and most sensitive HTS plate reader
Most flexible Plate Reader for Assay Development
Flexible microplate reader with simplified workflows
Upgradeable single and multi-mode microplate reader series