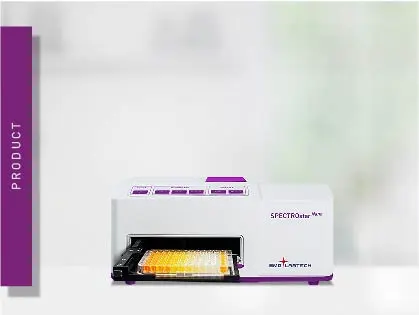
SPECTROstar Nano
Absorbance plate reader with cuvette port
Vaccines are powerful tools that have completely or nearly wiped out several viral diseases. Other infectious diseases however still await the development of effective vaccines. These diseases include malaria and HIV, whose vaccine development has been going on for decades. Presently, the globe is dealing with a pandemic of the novel Coronavirus (SARS-CoV-2) and the world is clamoring for an effective vaccine. This has spurred a rapid advancement of new vaccine technology into clinical trials and could very well lead to a new era in vaccine development.
Vaccine development and design have changed considerably since the initial discovery by Edward Jenner, but the principal underlying immunity remains unchanged. A traditional vaccine takes either a piece or the entire pathogen (albeit in a destroyed or weakened form) and is delivered to the immune cells via subcutaneous injection. The foreign invader is recognized as an outsider and the immune system initiates a memory response via T-cells and B-cells (fig. 1). Thus, a vaccine mimics the natural infection by a pathogen and induces the immune system to prepare for subsequent exposures. This can provide life-long immunity to some pathogens. Some viruses such as influenza can mutate often and rapidly, which prevents complete immunity, but a partial recognition is still sufficient to limit disease severity.
Vaccines confer a benefit to both individuals and populations. High rates of immunization within a population leads to what epidemiologists call herd immunity. Viruses need a population of naïve hosts to spread rapidly, thus herd immunity can prevent viral outbreaks. In many instances, disease burden from several vaccine-preventable pathogens has been reduced by over 99% since the introduction of childhood vaccination regimens1. Vaccines not only reduce morbidity but are also remarkably economical. Estimates suggest that direct intervention with vaccines save approximately US$ 14 billion each year in healthcare costs2.
Live attenuated virus vaccine
The original vaccine using live attenuated viruses dates back to the early work of Jenner and Pasteur on smallpox. The fundamental idea behind this approach is to mimic the natural course of infection and thus elicit a strong immunologic response. The attenuation process involves growing the virus on cells from a nonhuman species or at nonphysiologic temperatures for prolonged periods. The result is a severely weakened pathogen that does not induce clinical symptoms. It has the unique advantage of activating both humoral (antibodies) and cell-mediated immunity. Newer methodologies utilize reverse genetic approaches to produce attenuated viruses in the lab from cloned cDNAs. This is advantageous for seasonal pathogens such as Influenza. Once predominate strains have been sequenced, new vaccines can be produced to specifically target the current circulating viruses. One drawback to this method is a careful balance between efficacy and safety. Some attenuated pathogens could revert back to a pathogenic form and induce disease, although this has only been observed in one case involving the oral vaccine for polio3.
Inactivated vaccines
The second major approach to viral vaccines is to simply destroy or inactivate the pathogen. In most cases this is achieved with chemicals such as β-propiolactone or formaldehyde. While this approach allows full presentation of the virus to the immune system, it only confers immunity through neutralizing antibodies. Thus, this method is typically less immunogenic.
Subunit vaccines
The advent of recombinant DNA technology drastically altered the course of vaccine development. Scientists could now identify viral antigens and manufacture the subunit on a massive scale. This allowed development of vaccines for viruses that could not be grown in the lab, such as Hepatitis B Virus (HBV). In fact, the first commercially available vaccine using this technology was a recombinant vaccine targeting the HBV surface antigen. Newer recombinant technology uses antigens in the form of virus-like particles (VLPs). Think Lego pieces that self-assemble to form a replica of the virus. This has now been successfully used for the human papilloma virus and results in better immunity than natural infection4.
Nucleic acid vaccines
DNA and RNA vaccines provide the genetic information for antigens against which immunity should be conferred. This way, the vaccinated organism can produce pathogenic antigen on its own. In contrast to the use of attenuated and inactivated vaccines, there is no risk of infection. Furthermore, nucleic acids are cost-effective to produce and may be stored for a long time. The nucleic acid can be delivered to the nucleus (DNA) or cytoplasm (RNA) by means of gene delivery such as gene guns, liposomes, or nanoparticles. Even though, there is no nucleic acid vaccine approved for use in humans yet, several nucleic acid-based vaccines are in the phase I clinical trials for the new coronavirus (SARS-Cov2).
Regardless of the vaccine type, vaccine development follows a rigid process. Apart from the research needed for the development process, vaccine development includes regulatory approval, creation of production sites and implementation of quality control measures (fig. 2). Following, we will explain how research for a vaccine works and introduce individual methods that support the search for an effective vaccine.
Exploratory phase in vaccine development
Vaccine development starts in the exploratory phase. This first step takes typically between two and four years and is mainly conducted in academic labs. This phase intensively studies the pathogen. What is the structure of the pathogen, how does it enter cells, what are the receptors the pathogen employs to enter cells, how does the pathogen replicate and how does it cause disease? A second answer that needs to be answered in the exploratory phase is how it the immune system responds and whether the pathogen has mechanisms to evade the immune system5. Based on this information, an antigen is selected that can trigger an immune response and confer immunity.
Preclinical phase in vaccine development
Once a candidate is identified, preclinical testing proceeds in cultured cells and animal models. This step takes another two years. The preclinical vaccine development phase characterizes the vaccine candidate: how safe is it, does it confer immunity, is it responsible to use in humans, which dose can be used in a clinical trial? Thus, the transition into clinical phase is closely related to the data determined in preclinical phase.
Clinical studies
Clinical trials occur in 3 phases and takes up to 10 years. Phase I trial administers the vaccine candidate to a small cohort, usually less 100 individuals. The main goal is to confirm safety and efficacy. Phase II expands the candidate into a larger and more varied cohort. This phase also focuses more on dosing, method of delivery and immunization schedule. Phase III can include thousands or tens of thousands of test subjects, continues to measure the safety (rare side effects sometimes don’t appear in smaller groups) and broad immunogenicity.
In vitro tests used for vaccine development
While the characterization of the pathogen and search for a suitable antigen mainly rely on in vitro assays, the further the vaccine is developed, trials with animals and finally humans become vital. However, many assays are used throughout the vaccine development process. Here we present the most popular ones and explain why and when they are needed.
a) Interaction assays
Interaction or binding assays clarify whether molecules interact and the relative strength of this binding. They are used in the exploratory stage to study the binding of virus particles to membrane receptors. Further, they help refine the molecule with highest affinity to the antigen, which produces a better candidate for subunit vaccines. Interaction assays mainly employ two principles: fluorescence polarization or resonance energy transfer. Fluorescence polarization requires the binding of a small molecule to a big molecule. The difference in molecular weight changes the polarization of the emission of a fluorophore and thus indicates binding. Resonance energy transfer depends on the proximity of an energy donor and a fluorophore as acceptor, either of which is linked to one of the interaction partners. The donor can either be a fluorophore (FRET) or a luciferase (BRET). The principle of these interaction assays used to measure receptor binding is described in the application note "NanoBRET assay for monitoring of ligand binding to GPCRs in live cells" and "Protein-ligand binding measurements using fluorescence polarization".
b) Infection assays
Virus infection assays are employed to develop vaccines conferring immunity to viruses. They study the infection and sometimes propagation of viruses using pseudoviruses or recombinant viruses. These are equipped with a luminescent or fluorescent reporter gene and mixed with a cell culture. Infected cells express the luciferase or the fluorophore and are detected with a microplate reader. The principle was used by a research group in Oxford (UK) to characterize an Ebolavirus pseudotype. A viral gene was replaced with the fluorophore gene eGFP and the pseudotype served as tool to find inhibitors of viral entry to host cells6 and a first step in development of an Ebola vaccine.
c) Neutralization assays
Neutralization assays test whether vaccine candidates disable virus infection. Therefore, it proves effective immunity, a step required in the later stages of vaccine development: during the preclinical stage animal sera are tested for antibodies that are able to neutralize a pathogen and during clinical stages human sera are tested (see figure 2). The neutralization assay is based on infection assays and again uses recombinant viruses that express mainly luciferases upon successful entry into cells. Thus, addition of a substrate produces light, which can be directly correlated to viral infection. Neutralization assays preincubate the (pseudo)virus with sera of immunized animals or humans. If antibodies present in the sera bind and inactivate the virus, it cannot infect cell cultures and no light is emitted in the assay. (fig. 3)
d) ELISA assays
An Enzyme-Linked Immuno Sorbent Assay (ELISA) assay is a common tool to quantify specific proteins. It uses specific antibodies against the protein of interest in combination with an enzyme-mediated analysis step commonly resulting in an absorbance readout. The assay principle and tips for carrying out the experiment are outlined in our blog post “Optimizing your ELISA assays”. ELISAs are indispensable for each vaccine development step. In the exploratory phase, ELISAs quantify antigens that can be used as subunit vaccines. In the preclinical step, ELISAs quantify the immune response of animals or humans. To this end, a pathogen structure (e.g. bacterium-specific lipopolysaccharide or viral structural proteins) is coated onto a microplate and diluted serum of an immunized individual is added as a sample. If the vaccination led to production of antibodies specifically binding the pathogenic structure, the antibodies will bind it and are immobilized in the microplate well. A second antibody is subsequently added and specifically recognizes the vaccine-induced antibody in the well. It specifically detects the immunoglobulin type (e.g. IgG, IgA). This way, an ELISA used in the preclinical and clinical stages of vaccine development results in a quantification of a specific immunoglobulin that specifically binds a pathogenic molecule. This principle is also used to determine the antibody titer of immunized individuals.
e) Cytotoxicity assays
Vaccine candidates need to be tested for toxicity in the preclinical vaccine development stage. Initial testing is performed in in vitro cell cultures and may replace animal studies. Toxicity assay test if a vaccine candidate kills or harms living cells. A detailed description of available assays can be found in our blog post “Cytotoxicity – These assays tell you what your cells don’t like”.
f) Instrumentation for in vitro vaccine development assays
The assays employed during vaccine development range from biochemical to cell-based assays and produce either an absorbance signal, fluorescent signal or luminescent signal. A flexible multimode reader such as the CLARIOstar Plus detects all those signals and is further optimized to measure cell-based assays. An atmospheric control unit regulates CO2 inside the measurement chamber and thus allows to measure cellular infection in real-time as demonstrated in the application note Studying the molecular mechanism of viral replication in real time using the CLARIOstar Plus with ACU. Cellular assays needed for vaccine development also benefit from the possibility to scan the microplate well and record the signal at different positions of the well. This is important to correct for inhomogeneous distribution of cells in toxicity measurements as well as inhomogeneous infection across a microplate well.
The COVID-19 disease has upended the world and has reignited vaccine development. Scientists are hard at work developing new and novel ways to prevent additional COVID-19 outbreaks. The US based company Moderna Inc. has begun clinical tests on its mRNA vaccine (mRNA-1273)7 just 2 months after sequence identification. In this approach, the mRNA carrying the code for the spike protein of COVID-19 is delivered directly into our cells by a lipid nanoparticle. Our own cells will take up this nanoparticle, then produce the viral spike protein and trigger the immune system. This promising technology is stable, long-lasting, and early results suggest strong immune response. A similar approach uses an adenovirus vector8 to deliver the spike protein information to cells. Both options are like taking a different car to get to the same destination, so I guess we can call them the new rideshare vaccines.
Both preclinical and clinical vaccine research requires detection of antigen expression and neutralizing antibodies. BMG LABTECH microplate readers support vaccine development throughout the entire process.
Absorbance plate reader with cuvette port
Powerful and most sensitive HTS plate reader
Most flexible Plate Reader for Assay Development
Upgradeable single and multi-mode microplate reader series
Flexible microplate reader with simplified workflows
Pyrogen tests are vital to ensure the safety of different health interventions including pharmaceuticals, medical devices and an array of biological products. This blog looks at the different types of pyrogens as well as some of the widely used pyrogen tests.
Light scattering offers distinct advantages for scientists interested in immunology. Find out how the NEPHELOstar Plus is used for high-throughput immunological tests.
Read here, how BMG LABTECH microplate readers can help at advancing cancer immunotherapy research.